2.2 Renewable Energies
Site: | Hamburg Open Online University |
Course: | Green Hydrogen |
Book: | 2.2 Renewable Energies |
Printed by: | Gast |
Date: | Wednesday, 2 July 2025, 3:41 AM |
Description
This chapter discusses renewable electricity as the central raw material for green hydrogen production. It highlights the three origins of renewable energy: geothermal heat, solar energy, and gravity. Technologies such as photovoltaic systems, wind turbines, and hydropower plants are used to generate renewable electricity. Hydropower and biomass have limitations, making photovoltaic systems and wind turbines the main options for large-scale green hydrogen production.
Overview
Electricity from renewable energy sources is, along with water, the central raw material for the production of green hydrogen. In addition, the most important technologies used for this purpose will be briefly presented.
As the figure below shows, all renewable energy flows or energy sources that can be converted into electrical energy come from one of three origins. These three basic origins are geothermal heat, solar energy and the gravity between the celestial bodies, basically between the moon, the earth and the sun. The sun's radiation energy is not only an energy stream itself but is also converted into various other renewable energy flows and energy carriers through a variety of natural processes inside the earth's atmosphere. Examples of this are wind energy and biomass. In case of biomass, the radiation energy of the sun is converted into a usable energy carrier by the photosynthesis of plant organisms. Wind is an energy stream that occurs due to spatial differences in the atmospheric air pressure distribution, which, in turn, result from variations in solar radiation.
These different renewable energy streams can be used to generate electrical energy. A variety of different technologies, which are shown in the blue boxes in the figure, are used for this purpose. On a global level, the following technologies are of particular importance for the generation of renewable electricity:
- Photovoltaic systems
- Solar thermal power plants
- Wind energy turbines
- Hydropower plants
- Biomass power plants
Photovoltaic systems
Solar cells are the basis for generating electrical energy in photovoltaic (PV) systems and are able to convert the radiation energy of the sun directly into electrical energy. The basic principle for this conversion is the so-called photoelectric effect. In the photoelectric effect, the energy of photons, which can be imagined as the smallest units of light, is transferred to electrons. To ensure that this energy transfer can take place and finally lead to the generation of electrical energy, the material of the solar cells is crucial.
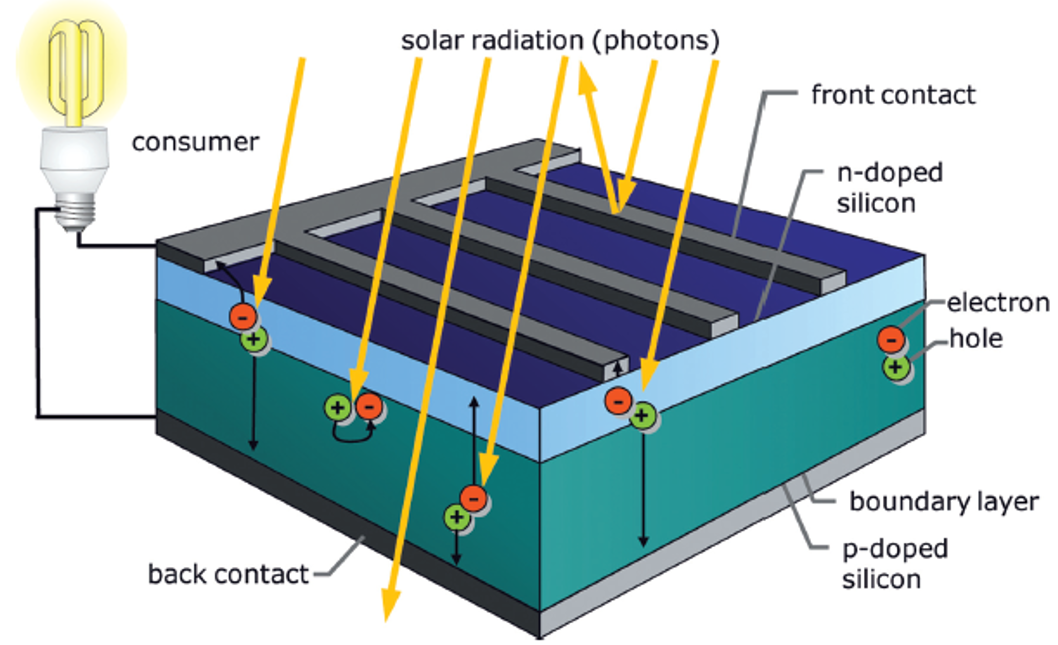
A central parameter for describing the technological performance of solar cells is the electrical efficiency. The electrical efficiency describes how much of the radiant energy arriving at the solar cell is converted into electrical energy. Currently, commercially available solar cells achieve an efficiency of 17 to 20 %. Most of the losses occur because only part of the incoming photon energy is transferred to the electrons due to physical limitations. Since solar cells generate direct current, but the electricity grid in Germany, as well as most consumers, need alternating current, PV systems are usually equipped with an inverter. The conversion in the inverter causes further losses, but these are small compared to the internal losses of the solar cell.
Wind energy turbines
Modern wind turbines slow down the wind and extract energy from the flowing air mass with the help of rotor blades. Wind turbines convert the kinetic energy of the wind first into mechanical energy (the rotation of rotor system) and then into electrical energy. However, it is physically impossible to extract all the energy from the wind: if we would extract all kinetic energy, the wind speed would become zero and then the path would be blocked by the non-moving air while the moving air would just go around the wind turbine so that no energy can be extracted any longer. Thus, a wind turbine can theoretically convert a maximum of 59.3 % of the wind power into mechanical power at the rotor. This ratio of extractable power to maximum wind capacity is described by the so-called Betz's coefficient.
- Rotor blades
- Rotor hub
- Generator
- Power train with brake (and gear)
- Wind sensors
- Yaw drive
- Nacelle
- Tower
- Foundation
- Grid connection
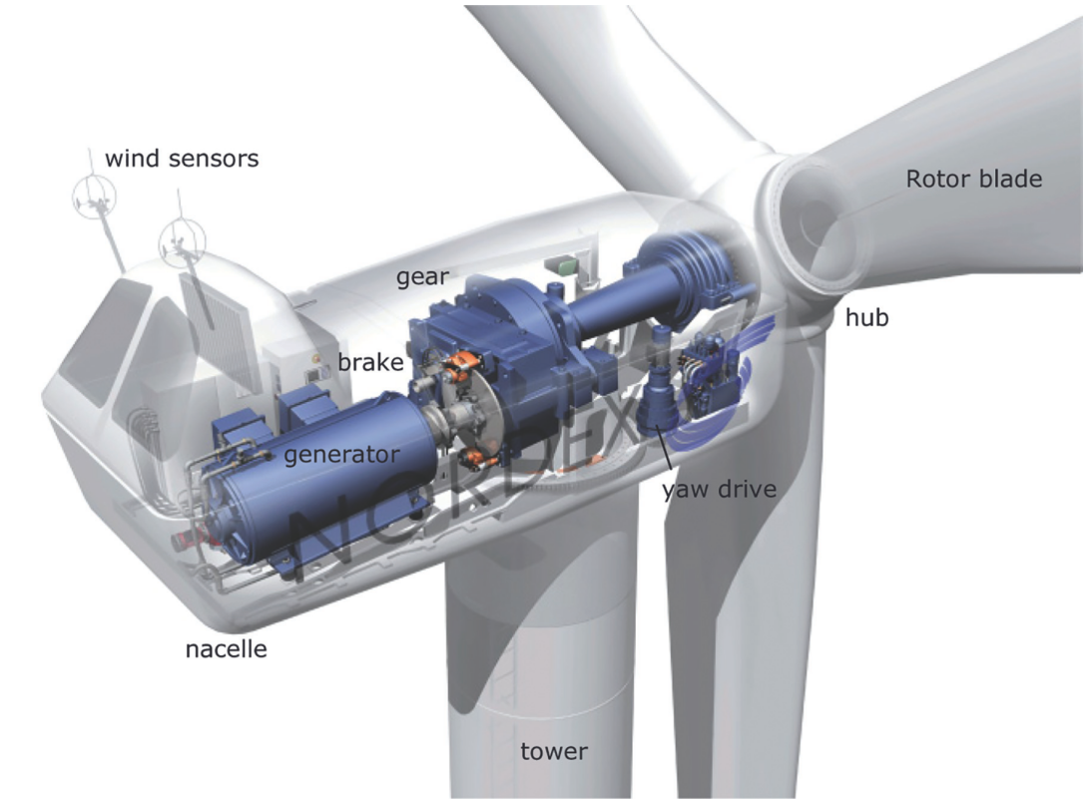
In addition to the limitation of the usable wind energy indicated by the Betz's coefficient, the energy conversion in the generator and, if present, the gear also lead to relevant losses. In the optimum case, currently available wind turbines can convert a maximum of around 45% of the wind capacity into usable electrical power.
The nominal power is an essential characteristic value for wind turbines and describes the electrical power output at the designated wind speed. In recent years, the nominal power of wind turbines available on the market has increased considerably. Meanwhile, turbines with a rated output of over 10 MW are being offered. However, turbines in the double-digit MW range are only installed offshore, i.e. at sea.
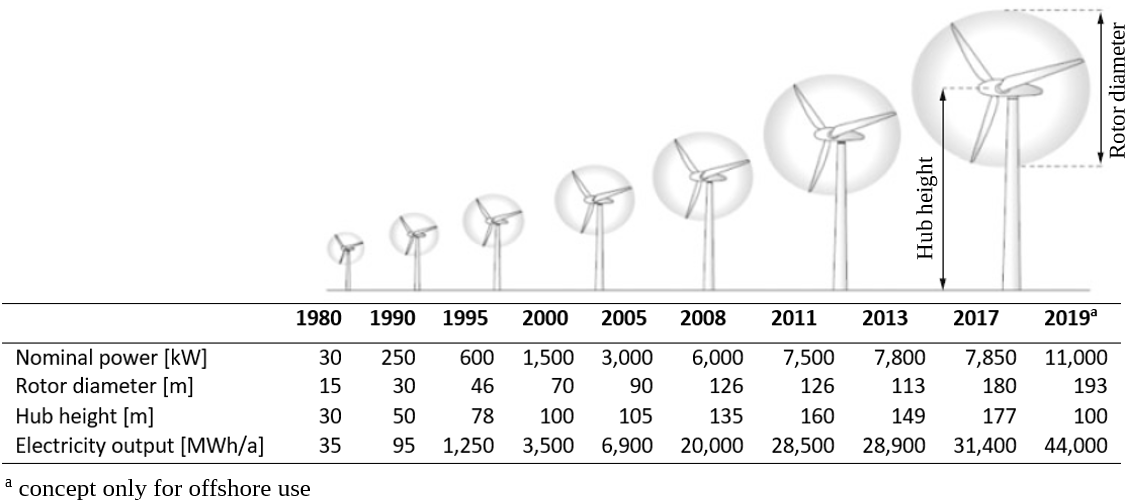